
Look up at the night sky and among the stars you might see a few brighter dots — the planets in our solar system. What you won’t see, though, are the most common kinds of planets in the Milky Way: super-Earths and sub-Neptunes.
These worlds, with sizes between that of Earth and Neptune, account for about one-third of known exoplanets. But they are absent from our own cosmic neighborhood. Why they don’t appear around the Sun is an intriguing question that astronomers are now working to answer.
Defining planets
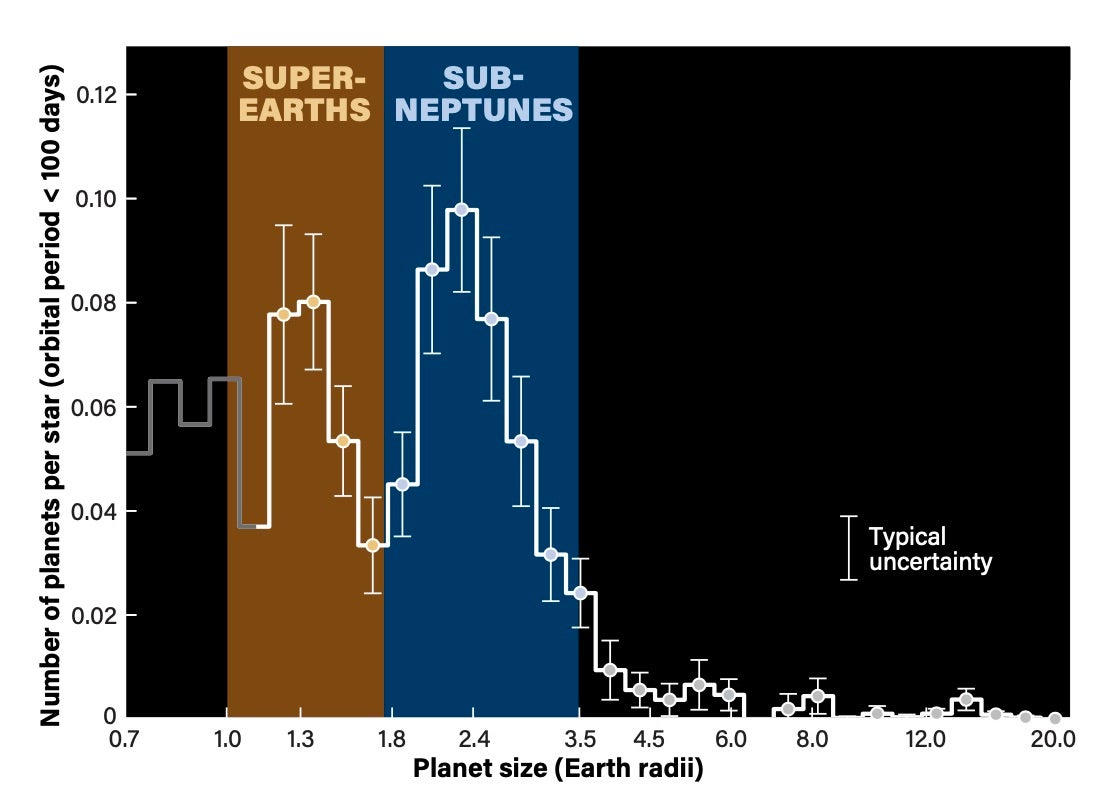
To explore this mystery, astronomers must understand what these planets are like and how they fit into the architecture of planetary and solar system formation.
Super-Earths and sub-Neptunes are worlds with two to 10 times Earth’s mass, and radii between those of our planet and Neptune. (Although more massive than Uranus, Neptune’s radius is roughly four times that of Earth, smaller than its fellow ice giant.) The terms super-Earth and sub-Neptune are sometimes used interchangeably, but many astronomers use the size of a planet’s atmosphere relative to its amount of rock to differentiate between them. Rocky planets up to 1.75 Earth radii with a relatively small atmosphere are considered super-Earths, while sub-Neptunes generally have radii between 1.75 and three times Earth’s, and host thick atmospheres with a smaller rocky core.
These definitions are still evolving, though. Johanna Teske of the Carnegie Earth and Planets Laboratory in Washington, D.C., says that researchers aren’t sure whether atmospheres are the best way to determine the two categories, or if other factors, such as core size, are also meaningful.
We do know that super-Earths and sub-Neptunes vary in composition, from rocky to icy to gaseous and anything in between. We know they can have atmospheres, though whether they might host oceans or moons remains the subject of speculation.
But these intriguing worlds do seem to be abundant.
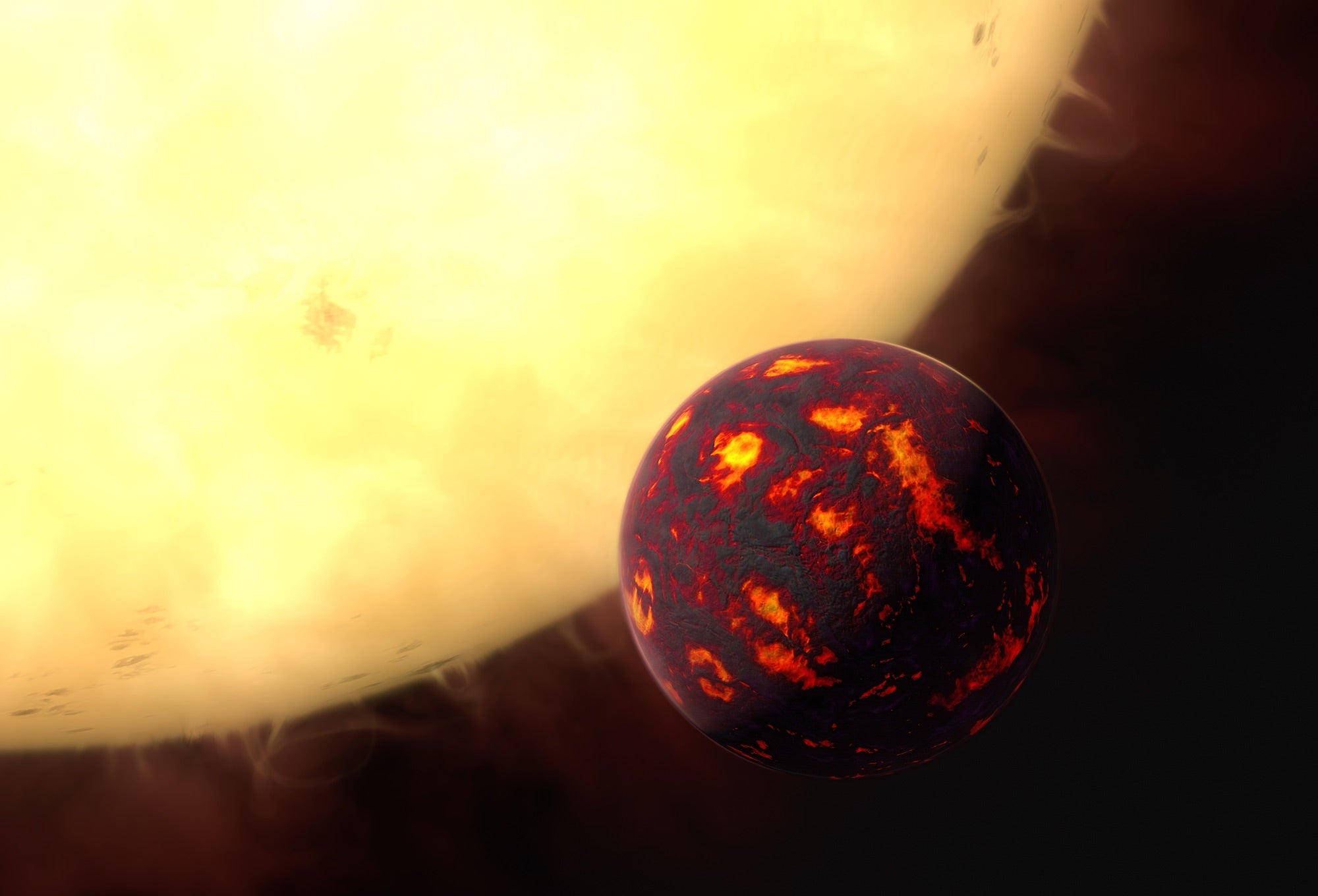
Finding other worlds
Although scientists long believed that exoplanets existed, it is only in the past three decades that technology has caught up with our desire for discovery. Astronomers have found thousands of exoplanets since the mid-1990s, with 1,661 classified as super-Earths as of this writing.
So, how do we discover them? Several methods exist.
All planets gravitationally interact with their star, as both star and planet orbit a common center of mass. Some planets have a large interaction — this happens when either the planet is massive, the distance between the two is small, or both. As it orbits, a planet pulls on its star, moving it slightly toward and away from Earth. Scientists see this “wobble” as a shift in the star’s light toward the blue or red end of the spectrum, respectively. Measuring this shift is known as the radial velocity method.
The radial velocity method is quite sensitive to hot Jupiters, massive planets orbiting close to their stars, because they induce a stronger wobble over a shorter period than do smaller planets farther away. Therefore, this method is biased toward hot Jupiters and less effective at finding super-Earths and sub-Neptunes — even “hot” (close-in) ones, which induce smaller signal amplitudes due to their smaller masses, Teske says.
Another method for finding planets is the transit method, in which astronomers measure the slight dimming of a star caused by a planet passing in front of it. Scientists can infer the size of the planet from the dip it causes in the light curve, or amount of starlight measured over time. This method is also most sensitive to larger planets close to their star because they block more starlight when they transit, causing a larger dip in brightness, and are also more likely to cross in front of their star from our point of view. The key is that the transit method requires that the planet and star be aligned along our line of sight so we can observe a transit. Because it is orientation-dependent, many systems are not discoverable this way simply because the planets don’t line up between Earth and the star they orbit.
In cases where both techniques can be used together, astronomers can measure a planet’s size from transits and its mass from radial velocity. This allows us to calculate the planet’s density and make better guesses about its composition — which, in turn, might hint at its habitability.
Planetary intrigue
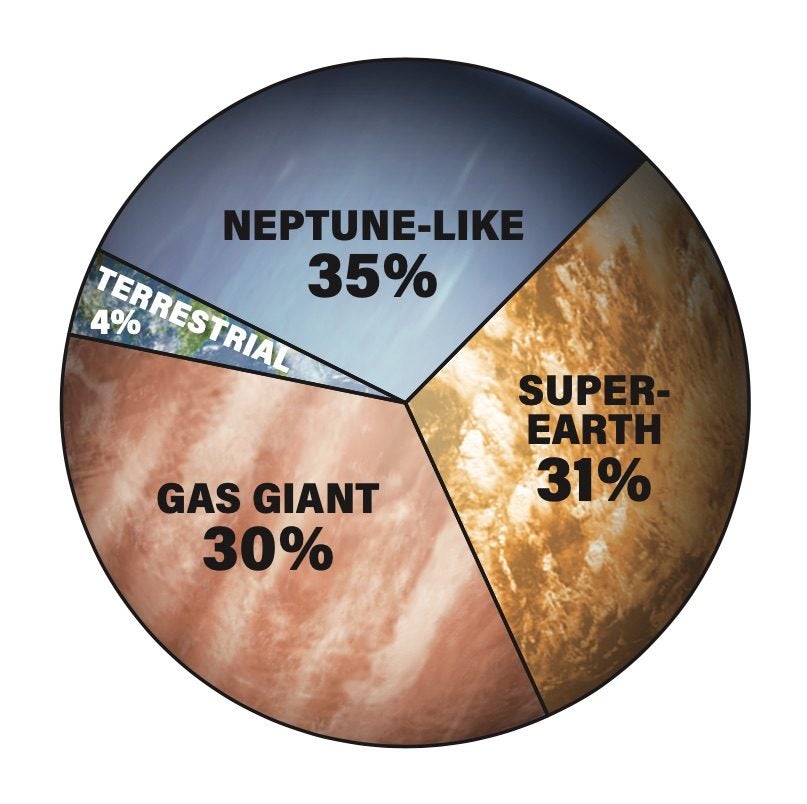
Why are we so fascinated with super-Earths? After all, Earth is the only planet we know of with life, so why not focus on finding and studying other small planets?
For most of Earth’s 4.5-billion-year history, macroscopic life did not exist. Changes in the Sun and geological activity created massive shifts in the planet’s climate over time, transforming it into one friendly to developing life. But these changes were not inevitable. They happened by pure chance.
Super-Earths and sub-Neptunes are appealing because they might offer an even better chance of developing habitable conditions — and inhabitants. That’s because many orbit M (red) dwarfs, a class of star with a much longer lifespan than our Sun, which gives more time for conditions to change and life to arise. M dwarfs outnumber Sun-like stars by several orders of magnitude, and almost half of M dwarfs surveyed so far have super-Earths.
Not only do many M dwarfs host super-Earths, but about 50 percent have super-Earths in their habitable zones, where temperatures are right for liquid water to exist on the surface. In our solar system, this is the region between the orbits of Venus and Mars. Given those statistics, astronomers consider the potential number of super-Earths in the habitable zone around stars in the Milky Way alone to be in the tens of billions. So, the search for super-Earths and sub-Neptunes is interesting to illustrate the way planetary systems form and evolve. But it’s also important for its potential implications for the search for life beyond Earth.
The TRAPPIST-1 system
One of the most interesting candidates for further study is TRAPPIST-1, a star system discovered in 2017 and located approximately 40 light-years away in the constellation Aquarius. Seven terrestrial planets orbit this star; several are super-Earths. JWST has just begun collecting data on these worlds, with recent measurements indicating that the innermost planet, TRAPPIST-1 b, does not have an atmosphere.
Although that seems like a blow to the system’s habitability, TRAPPIST-1 b in particular orbits the star too closely to be a viable candidate for hosting life. But three planets — TRAPPIST-1 e, f, and g — are in the star’s habitable zone and astronomers are eagerly awaiting observations of them by JWST.
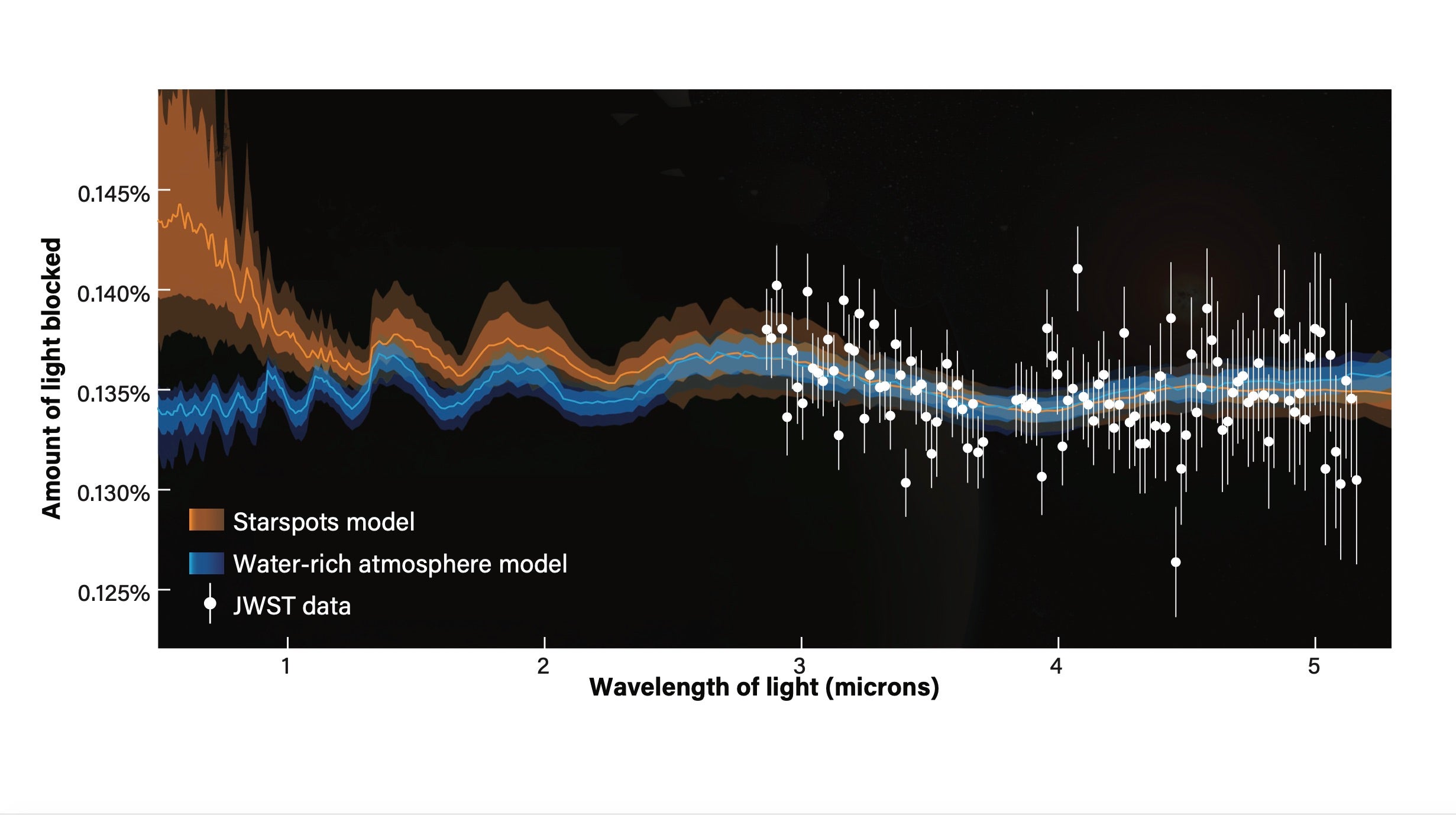
A breath of fresh air
In addition to finding new worlds, characterizing them is vital to learn whether they host conditions favorable to life. The James Webb Space Telescope (JWST), the largest and most powerful space telescope ever built, is partly dedicated to this task. With a mirror six times larger in area than that of the Hubble Space Telescope (HST), its instruments operate in infrared wavelengths. In addition to three cameras, JWST also hosts several other instruments, including multiple spectrographs and coronagraphs. The spectrographs disperse light by wavelength, allowing us to learn about the temperature and chemical composition of a planet. The coronagraphs block unwanted light from the host star so the planet’s light can reach the sensors for direct imaging.
With these tools, JWST can provide detailed observations of exoplanetary atmospheres. Scientists can now search for signatures of water, methane, oxygen, and other molecules that offer a look into the atmospheres of super-Earths and sub-Neptunes. These observations are charting the path toward the search for biosignatures, Teske says, which are spectral signs of past or present life. Although this search is starting with JWST, she adds, it will likely require even larger ground- and space-based telescopes to inform us about the true nature of such planets’ habitability.
Teske is part of a JWST program to better understand the atmospheres of super-Earth and sub-Neptune planets, called Compositions of Mini-Planets for Atmospheric Statistical Study, or COMPASS. “We are thinking of and want it to be a helpful pointer for the community as to what observations might be most useful or distinguishing moving forward” as more planetary atmospheres are probed, she says. At first, the COMPASS team is focusing JWST on 12 planets, with the aim of determining the best wavelengths to probe for hallmarks of habitability, such as water in a planet’s atmosphere.
“Before JWST, we had a few atmospheric observations of these types of small planets, mostly from HST, but not many,” says Teske. “At this point, the basic question of, ‘How does planet size correspond to the presence of an atmosphere?’ isn’t even well understood, so that’s something we will try to tackle with our program.” After that, she says, the team is eager to look at the diversity in atmospheric composition among these planets: “What are the relative abundances of molecules like water, methane, carbon dioxide, and what might that tell us about atmospheric processes or even interaction between the atmospheres and interiors of these planets?” she asks. “I’m particularly excited about that dimension — the atmospheres of small planets could be a new window into what is happening inside of them!”
The COMPASS program will also try to untangle how planets within the same system form. “We have four systems of sibling planets — two planets around the same star — in our program, so we can assess how similar their atmospheres are and, if they’re not similar, why that might be,” Teske says. Overall, she adds, the team hopes their results can be interpolated onto other super-Earths and sub-Neptunes, “hopefully starting to pick out trends between their atmospheric compositions and other parameters like bulk density or radiation from the host star.”
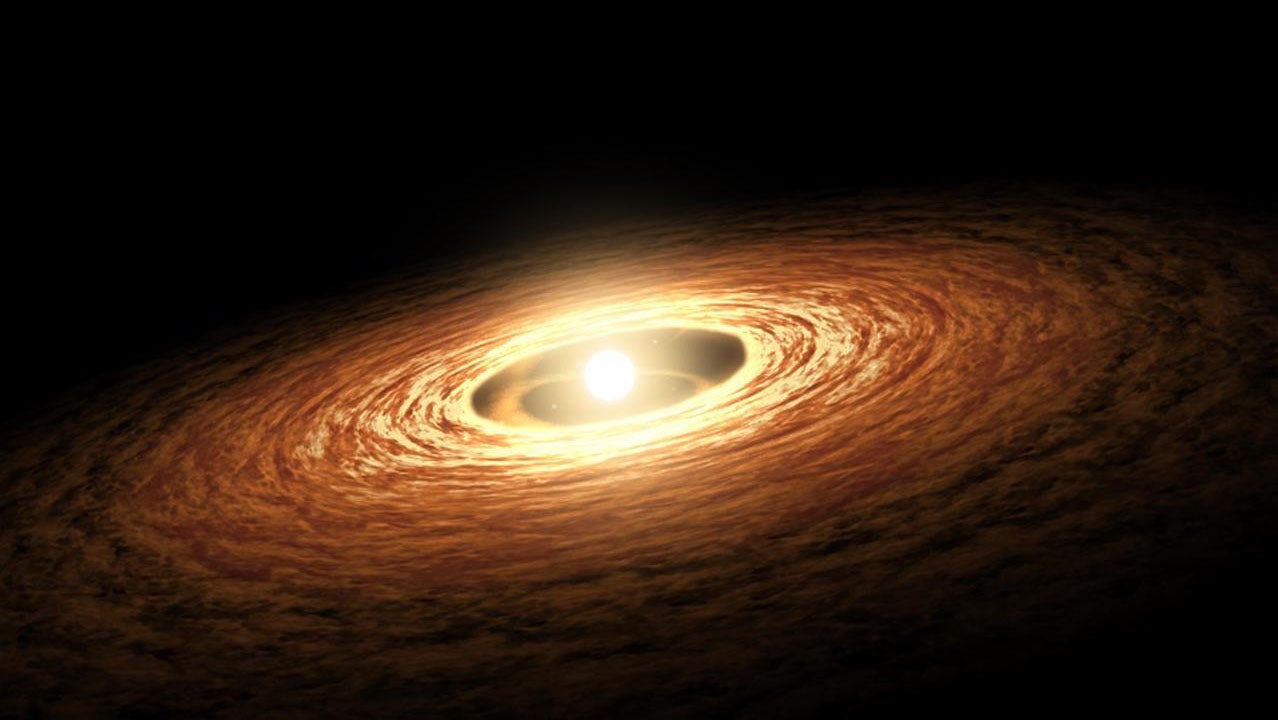
Creating worlds
Clearly, super-Earths aren’t hard to form. And the key to how these specific types of planets are created lies in understanding how all planets form.
Models show that the planets in our solar system grew by the gradual accumulation of material in the protoplanetary disk of dust and gas around the young Sun. Although the disk’s inner regions contained denser material, less of it existed close to the star, inside what astronomers call the ice line. This is the dividing line inside which temperatures are high enough to keep volatiles, such as water, in gaseous form. Beyond the ice line, cooler temperatures mean water and other compounds exist only as ice. So, the inner part of the disk produced smaller but denser, rocky planets: Mercury, Venus, Earth, and Mars. In the outer disk, material tended to accrete faster and produce more massive planets, whose gravity then pulled in nearby gas into thick atmospheres to form worlds such as Jupiter and Saturn. This is known as the core accretion hypothesis, the most widely accepted model for how our solar system formed.
It seems that one reason we don’t have any super-Earths is the existence of Jupiter, whose orbit is highly responsible for our solar system’s dynamics. Scientists believe Jupiter may have migrated toward the inner part of the solar system before moving back out to its current position. This movement would have destabilized the orbit of any objects it neared, resulting in a lot of material spiraling in toward the Sun. Known as the Grand Tack hypothesis, this reasoning could explain why little material would have been left to form a super-Earth in the Sun’s habitable zone.
But what if we did have a super-Earth? Stephen Kane, an astronomer at the University of California, Riverside, ran computer simulations to probe the effects of such a planet on our solar system. His experiment dropped a fully formed super-Earth into the modern-day solar system and ran time forward for 10 million years to see what would occur.
The results unequivocally showed that adding a super-Earth had devastating impacts on the orbital stability of the terrestrial planets. Although the gas giants Jupiter and Saturn were relatively undisturbed, changes in Jupiter’s orbit ejected some or all four rocky planets from the solar system; in some cases, even Uranus and Neptune were kicked out.
Kane found that reducing its mass and placing the super-Earth at 3 astronomical units from the Sun (1 astronomical unit is the average Earth-Sun distance) was the most stable option with the least influence on Jupiter’s orbit. But outside of this exact scenario, Kane noted in a press release, “things would go poorly. Despite many astronomers having wished for this extra planet, it’s a good thing we don’t have it.”
In other solar systems with suns like ours, Jupiter-like planets are rare and super-Earths are common. So, it seems that our solar system might not be a good model for developing a general theory of planetary system formation.
As scientists continued to discover exoplanets, they began noticing some trends: namely, that most super-Earths are rocky and when there are multiple super-Earths in a single system, they all have extremely similar features, such as size and mass. It’s as if there is a blueprint or mold for super-Earth formation. But why?
Again, it seems the key lies with Jupiter. In 2020, astronomers Konstantin Batygin and Alessandro Morbidelli proposed a new formation theory for Jupiter’s four largest moons, which are also similar in size to one another. Like the young Sun, Jupiter had a disk of material around it early in its life. The researchers showed that a balance in the forces pulling dust grains toward Jupiter and pushing them away formed a ring around the planet composed of grains with a certain size. These particles were the building blocks of the moons, which accumulated material until drag within the disk caused them to leave it and they stopped growing. This happened over and over again, creating multiple near-replicate bodies.
Since then, those same researchers have extended this theory to planet formation. They found that in protoplanetary disks, a similar ring forms where rocky material accumulates when forces are balanced. In systems with ample rocky material, their models show that as the rocky super-Earths reach a given mass, they move in toward the star and stop growing, and the process continues with the next planet. Their most recent results were published earlier this year in Nature Astronomy.
Although a nascent theory, this model has a lot of promise for explaining why we don’t have super-Earths in our solar system. Solar system architecture appears to be highly dependent on the amount of mass in that rocky ring. With little mass, we get a solar system that looks like our own terrestrial planets. A more massive ring spawns what seems to be homogenous super-Earths.
The search continues
The mystery of our solar system’s missing super-Earths and sub-Neptunes is not yet solved. Studying these exoplanets is one of NASA’s top priorities and JWST plays a pivotal role. This fascinating and challenging field of research is poised to reveal new insights into the formation and evolution of planets and solar systems, the conditions for habitability and life, and the diversity and complexity of the universe.